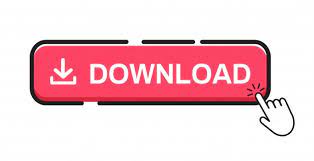
The microcavities were fabricated from graphite monocrystals, using lithography and dry etching, and had internal diameters of 1–3 μm and depth of ∼100 nm (“Methods”).
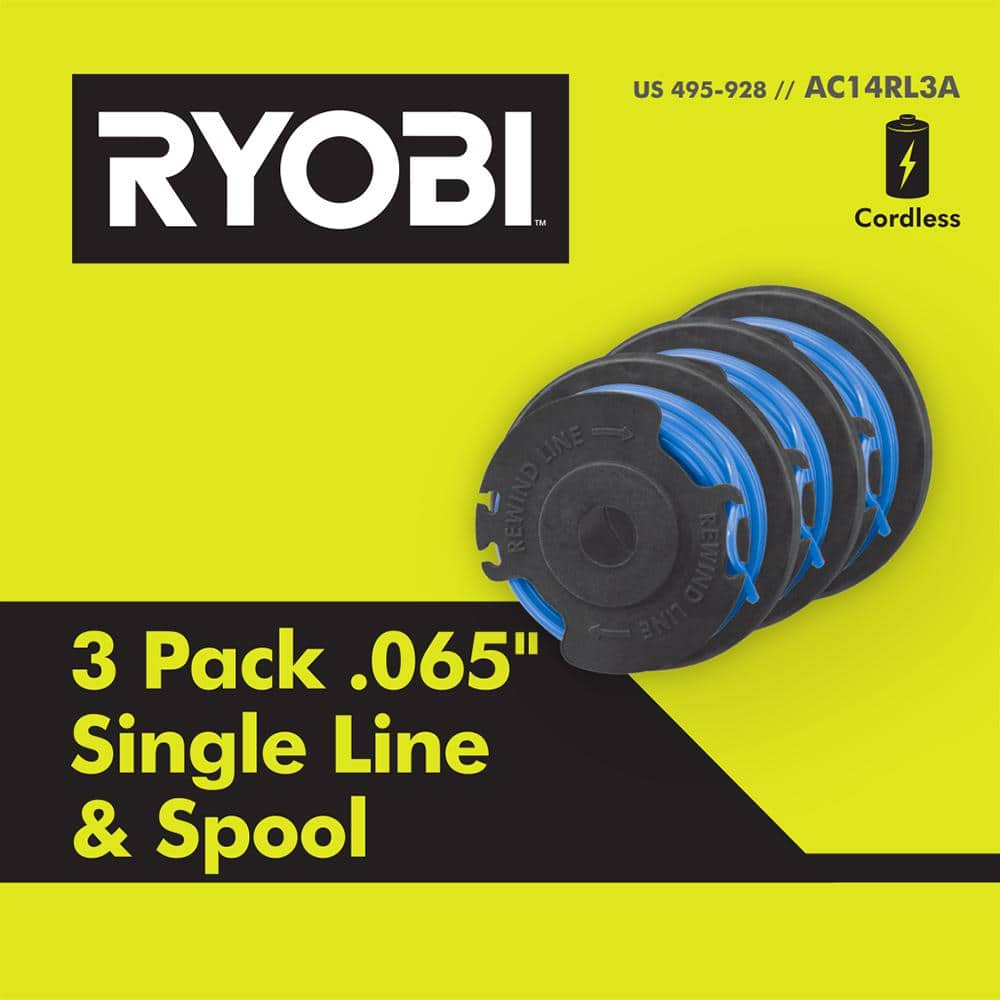
Our devices were micrometer-size cavities sealed with monolayer graphene (Fig. Gas permeation measurements reveal exponentially large selectivities with activation barriers that depend quadratically on gas molecules’ kinetic diameter. In this work, we achieve the activated regime by creating individual angstrom-scale pores in monolayer graphene by its short exposure to a low-energy electron beam. The experimental difficulties and lack of understanding are further exacerbated by prohibitive computational costs of simulating molecular permeation in the activated regime 17, 18, 19, 20. Indeed, even monovacancies in dichalcogenide monolayers were suggested to exhibit the conventional Knudsen flow 9. Little remains known about the latter regime, which has proven to be extremely difficult to reach in experiment 5, 8, 9. Still, this is many orders of magnitude smaller than S predicted for the activated-transport regime, d P < d K 1, 14, 15. For smaller pores with d P ≈ d K, S up to 10–100 have been reported for monolayer graphene 5, 8, and even higher selectivities ( ∼10 4) were found for some defects with an estimated diameter of ∼3.5 Å in bilayer graphene 4. Experimental clarity has so far been achieved only for the classical regime of d P > d K where the flow is governed by the Knudsen equation, and the resulting modest selectivities arise from differences in thermal velocities of gases having different molecular masses m 7, 8, 9, 16. At present, this optimistic assessment is based mostly on theoretical modeling. This unique combination of material properties holds a promise of better selectivity-permeability tradeoffs than those possible by conventional membranes 3, 13. On the other hand, angstrom-scale pores with effective sizes d P smaller than the kinetic diameter d K of molecules should pose substantial barriers for their translocation, which is predicted to result in colossal selectivities S > 10 10, even for gases with fractionally ( ∼25%) different d K such as, for example, H 2 and CH 4 1, 14, 15. On one hand, the atomic thickness of 2D materials implies a relatively high permeability as compared to traditional 3D membranes.
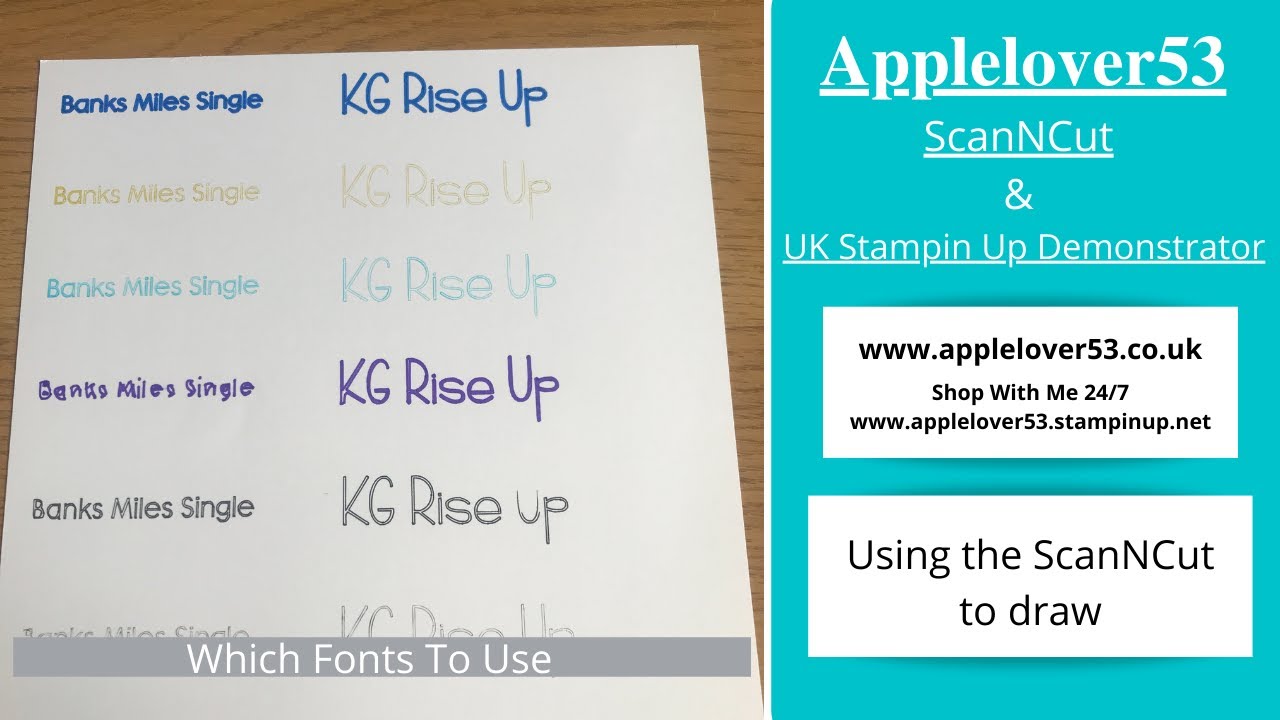
Interest in angstroporous 2D materials is strongly stimulated by potential applications, particularly for gas separation as an alternative to polymeric membranes employed by industry 3, 13. Two-dimensional (2D) membranes with a high density of angstrom-scale pores can be made by engineering defects in 2D crystals 1, 2, 3, 4, 5, 6, 7, 8, 9 or, perhaps more realistically in terms of applications, by growing intrinsically porous crystals such as, e.g., graphynes 10, 11, 12.
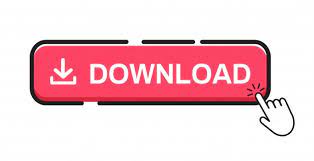